Are three doses of stereotactic ablative radiotherapy (SABR) more effective than 30 doses of conventional radiotherapy?
Introduction
Lung cancer continues to be the leading cause of cancer-related mortality in men and women, whereby the rates recently showed a significant decline in women after continuously increasing since the 1930s (1). For stage I non-small-cell lung cancer (NSCLC), surgery alone results typically in a 5-year overall survival (OS) of 60-70% (2).
For patients with stage I NSCLC who cannot undergo radical resection e.g., for medical reasons, definitive radiation therapy is the appropriate alternative to surgery. For these patients standard fractionated radiotherapy alone can lead to a 5-year OS of 8-15% and a cancer specific survival rate in the range of 30-55% (due to competing risks of death) (3,4). Recent publications also support the strategy of treating only the primary tumor and PET positive lymph nodes (involved node radiotherapy; INRT) instead of treating the elective nodal regions (elective nodal irradiation; ENI) to reduce the volume of irradiated healthy tissue especially keeping the volume of lung that receives more than 20 Gray (Gy) below 35% (using conventional fractionation) (5-8). However, local tumor control after a conventional treatment with 55 to 70 Gy delivered over 4 to 7 weeks is as shown above suboptimal. Available data suggest that conventionally fractionated doses of >>70 Gy might be necessary to control >90% of tumors locally (9-11).
Already in 1995, Blomgren et al. showed improved local control rates for patients with lung cancer treated with stereotactic hypofractionated radiotherapy compared with conventionally fractionated radiation (9). After a phase of careful dose escalation, single dose treatments at doses of 30 Gy (12) and fractionated treatments with 5×10-12 Gy or 3 times 20 Gy are currently applied on various institutional protocols and seem to be reasonably safe, with increase of the tumor control (SBRT prescription of 60 Gy in 3 fractions equates to as much as 150 Gy delivered in conventional fractions) (10,11,13).
The aim of this review is to summarize the updates of the radiobiology, technical aspects and clinical outcomes of SBRT.
SABR/SBRT
Stereotactic body radiation therapy (SBRT) or SABR (stereotactic ablative radiotherapy) is a relatively novel concept in which high doses of radiation are directed focally onto malignant lesions in organ sites other than the brain, including lung, liver, and spine tumors. The idea of SBRT is derived from the experience in treating metastatic lesions in the brain by SRS (stereotactic radiosurgery). In SRS very high radiation doses are delivered to small brain lesions in a single session, with the intent to ablate all malignant tumor cells in one setting. The success rates of this treatment approach, with local tumor control rates as high as 93.3%, have made SRS a standard of care for limited metastatic disease to the brain (14-16).
SBRT as discussed here will largely adhere to the accepted definition in the United States as the delivery of high-dose focused radiation in one to five fractions onto small malignant lesions. High-dose delivery is most often understood as single fraction doses exceeding 5 Gy. Small lesions are most often defined as being less than 5 cm in maximum diameter. Focal radiation delivery refers to narrow planning target volume (PTV) margins added to a target volume delineated in consecutive slices of a CT, MRI or PET radiation planning dataset. Additionally, SBRT is a radiation therapy modality for which a target has to be directly or indirectly localized before the radiation dose is delivered.
Steep dose gradients between the lung lesions and surrounding normal tissue are a hallmark of SBRT dose distributions, and achieve excellent normal tissue sparing. This is accomplished by using multiple radiation beams which are shaped according to the tumor outline, and are all centered upon the lesion. While each of the radiation beams delivers a small fraction of the cumulative radiation dose, the dose at the target, where all radiation beams intersect, is summing up to high tumoricidal dose levels. Similar dose concentration can be achieved using arc delivery techniques during which a multi-leaf collimator, a radiation beam shaping device, continually adjusts the radiation port to the shape of the target from a given beam's eye view. SBRT radiation plans use 7 to 11 individual radiation beams arranged coplanar or non-coplanar around the target lesion, with little incremental plan quality gained when the number of radiation ports exceeds 9 (17-21).
Extracranial stereotactic radiotherapy poses several challenges for patient immobilization and tumor localization. Framed and frameless systems have been developed for this purpose. In 1995, Blomgren et al. described a technique of SBRT using a custom-made body cast and stereotactic coordinates (22). Lohr et al. introduced in 1999 a body cast and head mask system with a stereotactic body frame for patients with paraspinal tumors in the thoracic and lumbar spine, the same group treated also liver tumors with single dose stereotactic irradiation using a vacuum pillow and an abdominal compression. Both groups reported an acuracy of ≤5 mm (23,24). For the lung, Uematsu et al. introduced 2001 a frameless approach using the FOCAL unit, a combination of linac, kV simulation and a CT-scanner. Breathing with an oxygen mask and an abdominal compression belt allowed the intrafractional tumor motion to be less than 5 mm (25).
The growing interest in SBRT has been driven by advances in the radiotherapy planning imaging techniques and delivering techniques, which allow increasing treatment precision (26). A matter of concern by the use of SBRT technique in the treatment of lung tumors is their potential susceptibility to breathing-induced target movements, which might lead to dosimetric uncertainty and discrepancies between planned and delivered doses. This problem is a major concern for all tumor sites in the thorax (27). In 50% of lung tumors, a movement of 0.5 to 1 cm is observed, in 10% of more than 1 cm (28). While a broad spectrum of movement patterns is observed, by far the predominant direction of movements is longitudinally in the cranio- caudal direction (29). One solution of the breathing motion problem is the use of four-dimensional computed tomography (4D-CT) scans that correlate CT images with respiratory phases, allowing the visualization of the tumor motion (30). The concept of gating uses this information, and will enable radiation beam delivery only when the lung, and thus the target, are in a defined proportion of the breathing cycle (31).
Real-time target tracking (continuous adapting of the radiation beam to the tumor position) or positioning with a fully robotic patient positioning system with six degrees of freedom are methods used to reduce the treatment margins but are not widely clinically implemented (32-34).
Another approach is the use of the breath-hold and respiration-synchronized gating. A breath-hold technique using ABC© (Active Breathing Control, Elekta, Crawley, UK) has been shown to be an accurate and clinically useful tool. It has the advantage of reducing the target motion such that the gross tumor volume (GTV) resembles a primarily static tumor on the planning CT scan. Intra- and interfractional reproducibility of this system is 1.7 and 3.7 mm (35-37).
Clinical experience and toxicity
One of the first SBRT trials for patients with medically inoperable NSCLC was the trial of the University of Indiana. The group recently updated the results of the phase II study. A total of 70 medically inoperable patients were included in the study. The SBRT treatment dose of 60-66 Gy was prescribed to the 80% isodose volume in three fractions. Median follow-up was 50.2 months and the local control at 3 years was 88.1%. Median survival was 32.4 months, the 3-year overall survival was 42.7% and the cancer-specific survival at 3 years was 81.7%. There was no difference in local control or survival between the T1 and T2 tumors, by tumor volume or by peripheral or central location. Grade 3 to 5 toxicity occurred in 5 of 48 patients with peripheral lung tumors (10.4%) and in 6 of 22 patients (27.3%) with central tumors (38).
The Scandinavian group also updated their results from a phase II trial. They treated 57 patients with NSCLC with SBRT with 15 Gy times three with a dose prescription at the 67% isodose of the planning target volume. Overall- and cancer-specific survival at 1, 2, and 3 years was 86%, 65%, 60%, and 93%, 88%, 88%, respectively. There was no statistically significant difference in survival between patients with T1 or T2 tumors, but the estimated risk of all failure (local, regional, or distant metastases) was increased in patients with T2 (41%) compared with those with T1 (18%) tumors. Local control at 3 years was 92%, local relapse was observed in four patients (7%) (39).
In 2006, Timmerman et al. reported the results of 70 patients treated with SBRT with a dose of 60 to 66 Gy total in three fractions during 1 to 2 weeks. The 3-month major response rate was 60%, the local control at 2 years was 95%. Median overall survival was 32.6 months and 2-year overall survival was 54.7%. Grade 3 to 5 toxicity occurred in a total of 14 patients. Among patients experiencing toxicity, the median time to observation was 10.5 months. Patients treated for tumors in the peripheral lung had 2-year freedom from severe toxicity of 83% compared with only 54% for patients with central tumors (40).
A group from Japan, reported their results of another phase II trial. They performed SBRT for 31 stage I NSCLC patients. SBRT was administered as 45 Gy in 3 fractions, however, when the tumor was close to an organ at risk, 60 Gy in 8 fractions were used. The doses were prescribed at the center of the tumors. The 3-year local control rates of T1 and T2 tumors were 77.9% and 40.0%, respectively. The 3-year overall and cause-specific survival rates were 71.7% and 83.5%, respectively. Five patients developed acute pulmonary toxicity ≥ grade 2 (41).
Ricardi et al. published in 2010 the final results of the phase II trial, where they included 62 patients with stage I NSCLC and treated them with three fractions of 15 Gy each, given every other day during a 1 week time, up to a total dose of 45 Gy. The dose was prescribed to the 80%-isodose encompassing planning target volume. At 3 years, local control rate was 87.8%, cancer-specific survival 72.5%, overall survival 57.1%, the majority of patients did not experience any toxicity; mild skin reactions, fatigue, dyspnea/cough or transient thoracic pain were recorded in approximately 10% of patients (42).
Recently, Timmerman et al. published the results of the RTOG 0236 trial. A total of 59 patients accrued, of which 55 were evaluable (44 patients with T1 tumors and 11 patients with T2 tumors) with a median follow-up of 34.4 months. The 3 year primary tumor control rate was 97.6%, the 3-year rate of disseminated failure was 22.1%. The rates for disease-free survival and overall survival at 3 years were 48.3% and 55.8%, respectively. The median overall survival was 48.1 months. Protocol-specified treatment-related grade 3 adverse events were reported in 7 patients, grade 4 adverse events were reported in 2 patients. No grade 5 adverse events were reported (43). Table 1 summarize the results of the prospective trials of the SBRT for patients with early stage NSCLC.
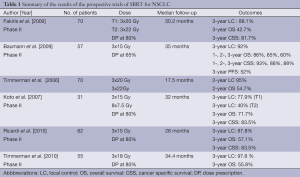
Full table
In a study, accomplished in our department, we included patients with 50 lung lesions who were treated with image-guided breath-hold SBRT with a regimen of 60 Gy in five fractions (Figure 1). Breath hold was performed with Active Breathing Control (ABC®, Elekta). Two year overall survival rate was more than 40%. 2-year local control rate was more than 80% without significant toxicity (44).
As shown above, the local control rates are more than 90%, when total doses from 54 to 60 Gy in three fractions are used. Currently, the RTOG 0915 trial is comparing two different SBRT fractionation schedules: 34 Gy in 1 fraction vs. 48 Gy in 4 fractions for patients with peripheral stage I NSCLC. The fractionation that proves to be less toxic, will be compared to the SBRT treatment schedule recommended by the RTOG (54 Gy in 3 fractions) in a phase III RTOG trial. The Dutch trial ROSEL (Radiosurgery or Surgery for Early Lung cancer) will compare surgery and SBRT for patients with stage I NSCLC. Primary objectives of the trial will be the local and regional tumor control, quality of life and treatment costs of 2- and 5–years. A similar study is the STARS (Stereotactic Radiosugery vs. Surgery) trial, which is comparing surgery and radiosurgery with CyberKnife. Endpoint is the overall survival at 3 years.
High single doses radiation therapy of lung tumors is a special challenge because of diverse reasons such as the highly volume dependent radiosensitivity of the healthy lung tissue and surrounding organs at risk (oesophagus, heart), the breathing-induced motion of pulmonary targets and the dosimetrically difficult situation of a soft-tissue lesion surrounded by low-density lung tissue.
Besides the vital organs at risk heart and oesophagus, the lung itself is one of the most radiation-sensitive organs with two distinctive manifestations of radiation damage with different time frames. As a severe early (subacute) side effect of radiation therapy, pneumonitis occurs in 5-15% 4-6 weeks after conventionally fractionated large-volume thoracic irradiation. Symptoms include dyspnoe upon activity, cough and subfebrile temperatures. The incidence of radiation pneumonitis depends on the radiation dose and the irradiated volume of the normal lung tissue (45). As a late side effect and consequence of radiation pneumonitis, pulmonary fibrosis may arise, rendering the affected tissue without function.
Tumor location is a well known predicting factor, which predicts severe toxicities, when using SBRT (54-60 Gy in 3 fractions) for patients with stage I NSCLC. Voroney et al. reported chest wall pain and rib fractures in patients with peripheral lung lesions (46). There is also a higher incidence of severe toxicities, when treating patients with 60 Gy in three fractions with central lung tumors, adjacent to mediastinal structures, defined as within 2 cm of the proximal bronchial tree, brachial plexus, or vertebral body. It seems, that in this cases, it is safer to apply the dose in more than 3 fractions (for example 12 times 5 Gy, 8 times 7,5 or 60 Gy in 4-5 fractions) (40,47,48).
Biological rationale
Over the last decades new insight into biological effects of irradiation in tissue has led to a paradigm change. The single target cell theory and solely cell kill from DNA damage is not sufficient to explain the complex biological effects of radiation. Furthermore, and specifically in SABR, the linear quadratic model-which is based on this rather simple theory and obtained from in vitro studies-may not be appropriate to assess in vivo data from SABR studies (49).
The importance of the tumor-microenvironment, the cross talk of malignant cells and host cells, the tumor bed effect, and the importance of other target cells than cancer cells are focus of intense ongoing research.
In addition, the radiobiology behind the effectiveness of high single doses or a small number of fractions of radiation may be very different from that underlying conventional fractionated non-ablative radiotherapy.
In this respect, endothelial cell apoptosis and micro-vascular dysfunction is observed only after high single doses of at least 8-10 Gy contributing significantly to tumor cell lethality and tumor cure by SABR (50-53). Although, the underlying mechanism of successive tissue damage and conversion of sub-lethal damage in tumor cells to lethal damage is not clear, it might involve leakage of circulating factors, a bystander effect of endothelial damage and ischemia induced complex cellular and molecular signalling.
In this respect, SABR might improve the elimination of potentially existent radio-resistant tumor stem cells which is a prerequisite for cancer cure. Tumor stem cells are thought to have better DNA repair mechanisms (54,55). In between fractions of conventional radiotherapy tumor (stem) cells try to repair sub-lethal damage, they can proliferate and transform into even more radio-resistant cells which possibly might facilitate tumor cell spread. After SABR and consequent micro-vascular dysfunction less repair and even direct apoptosis of cancer stem cells is conceivable. Ch'ang et al. observed stem cell apoptosis after single doses of 17Gy and higher (56).
In summary, endothelial cells and cancer stem cells may require a threshold dose to be crossed before their death is triggered which only can be accomplished by SABR.
Another aspect refers to radiation induced inflammatory and immune response. Radiation-induced inflammatory cytokine production is generally considerably stronger at higher radiation doses. It seems likely that dose fractionation may minimize the damage that results from this source by allowance of tissue repair.
Although likely to generate more inflammation, high local doses may be superior at generating "danger" signalling and rapid cell death and promoting anti-tumor immunity (57,58). Radiation induced massive tumor cell death by SABR leads to release of HMGB1 proteins among others from dying cells. Next to other proteins HMGB1 acts as a danger signal, a so called endogenous damage-associated molecular pattern (DAMP). Dying cells are phagocytosed by immature dendritic cells (DCs). DAMP signal through toll like receptors (TLR4 and TLR2) and are mandatory for host dendritic cells to mature (59). As a result antigen presenting cells (APCs) develop as targets for antigen specific CD8+ cells leading to a specific immune response.
As for apoptosis, it was shown that an immune response was optimal after doses of 8-10 Gy and higher.
Altogether, there are several biological aspects that might explain the excellent clinical tumor control rates after SABR.
Technical advancement
Accurate dose delivery to the patient is of utmost importance in external beam radiotherapy (EBRT) particularly if hypofractionated treatment techniques and/or dose escalation are used as in stereotactic body radiation therapy (SBRT). A precise dose calculation, delivery with steep dose gradients between tumor and healthy tissue and accurate tumor localization is essential.
In the last few years a lot of new methods and techniques were introduced in radiotherapy including intensity-modulated delivery (IMRT) with its steep dose gradients. IMRT offers the possibility to shape the dose distribution exactly around the target structures while organs at risk (OAR) are mostly spared. The full potential of IMRT can only be used when safety margins around the targets are reduced.
Image-guided radiotherapy (IGRT) offers the potential to reduce planning margins due to exact patient positioning and thus further helps to increase the therapeutic window (60-62). It has become clinical practice to re-position the patient according to 3D imaging data. To detect internal misalignment of organs relatively to the bony structures, it is preferable to use an image guidance system which is able to discriminate soft tissues. The common systems which can be used for online position correction are CT-on-rails (63), conebeam CT (CBCT) (Figure 2) (64-66), ultrasound (67,68) or electromagnetic signals (69). The positive effects of IGRT on the therapeutic dose distribution and the additional imaging dose have already been analysed (70-72).
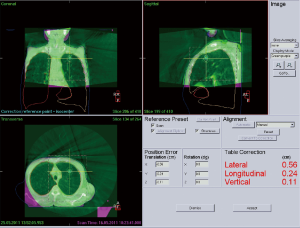
An exact image guided (or stereotactic) positioning of the patient is rather easy to achieve in rigid structures such as the skull but is a major challenge for mobile organs. Tumors in the thorax and abdomen can move significantly with respiration. This has to be taken into account during the treatment planning process and treatment delivery (73). Several approaches use four-dimensional (4D) CT- or CBCT-datasets, that allow the determination of tumor position in different breathing phases (74-76). However, sometimes the increased time for processing the large amount of data and also the extended and more complex workflow limit the usability in clinical routine (62,74). In contrast to those approaches breathhold techniques (77) have been suggested to reduce the effect of breathing motions while maintaining tight PTV margins around the tumor. With inspiration-breathhold treatment techniques ITV and thus PTV margins can potentially be reduced and doses can be escalated resulting in better tumor control rates. In addition, the total irradiated lung volume and, importantly, lung mass can be kept to a minimum (77-80).
Up to now long image acquisition times of ~60-120 s (kV-CBCT) limit the number of patients who can undergo volume image guidance under breathhold to eliminate motion artefacts. Therefore a new approach was suggested to combine the kV and the MV source of the linac for a simultaneously acquired, fast (~15 s) and accurate kVMV-CBCT reconstruction for image guided patient positioning. kVMV-CBCT based on a standard linac is promising and can provide ultra-fast online volume image guidance with low imaging dose and sufficient image quality for fast and accurate patient positioning for patients with lung cancer under breathhold (81,82).
Conclusions
Highly conformal body-stereotactic treatment with only a few or even single fractions has been successfully applied to extracranial lesions such as lung tumors, achieving high local control rates of more than 90% (97-98% at 3 years). The recommended from the RTOG fractionation schedule of 3 times 18 Gy, can be safely applied, whereby in patients with central tumors it is recommended to use more than 3 fractions (for example 5×12 Gy) to avoid severe toxicities. Additionally, despite comorbidities, SBRT is well tolerated even in patients with lower performance status, due to the less number of fractions (3 to 5). Breathhold techniques can help to reduce the effect of breathing motions while maintaining tight PTV margins around the tumor. During imaging they help to hold the tumor in a quasi stable position and thus reduces motion artefacts to a minimum. However, Long image acquisition times of currently ~60-120 s limit the number of patients who can be imaged with standard on board kV-CBCT for patient positioning at the linac during one breathhold phase. With the implementation combined kVMV-CBCT in the future, a shortening of the imaging time to ~15 s can be expected.
Acknowledgements
Disclosure: The authors declare no conflict of interest.
References
- Siegel R, Ward E, Brawley O, et al. Cancer statistics, 2011: the impact of eliminating socioeconomic and racial disparities on premature cancer deaths. CA Cancer J Clin 2011;61:212-36. [PubMed]
- Arriagada R, Bergman B, Dunant A, et al. Cisplatin-based adjuvant chemotherapy in patients with completely resected non-small-cell lung cancer. N Engl J Med 2004;350:351-60. [PubMed]
- Kupelian PA, Komaki R, Allen P. Prognostic factors in the treatment of node-negative nonsmall cell lung carcinoma with radiotherapy alone. Int J Radiat Oncol Biol Phys 1996;36:607-13. [PubMed]
- Sibley GS, Jamieson TA, Marks LB, et al. Radiotherapy alone for medically inoperable stage I non-small-cell lung cancer: the Duke experience. Int J Radiat Oncol Biol Phys 1998;40:149-54. [PubMed]
- Fernandes AT, Shen J, Finlay J, et al. Elective nodal irradiation (ENI) vs. involved field radiotherapy (IFRT) for locally advanced non-small cell lung cancer (NSCLC): A comparative analysis of toxicities and clinical outcomes. Radiother Oncol 2010;95:178-84. [PubMed]
- Slotman BJ, Antonisse IE, Njo KH. Limited field irradiation in early stage (T1-2N0) non-small cell lung cancer. Radiother Oncol 1996;41:41-4. [PubMed]
- Sulman EP, Komaki R, Klopp AH, et al. Exclusion of elective nodal irradiation is associated with minimal elective nodal failure in non-small cell lung cancer. Radiat Oncol 2009;4:5. [PubMed]
- Krol AD, Aussems P, Noordijk EM, et al. Local irradiation alone for peripheral stage I lung cancer: could we omit the elective regional nodal irradiation? Int J Radiat Oncol Biol Phys 1996;34:297-302. [PubMed]
- Blomgren H, Lax I, Näslund I, et al. Stereotactic high dose fraction radiation therapy of extracranial tumors using an accelerator. Clinical experience of the first thirty-one patients. Acta Oncol 1995;34:861-70. [PubMed]
- Timmerman R, Papiez L, McGarry R, et al. Extracranial stereotactic radioablation: results of a phase I study in medically inoperable stage I non-small cell lung cancer. Chest 2003;124:1946-55. [PubMed]
- Fowler JF, Tomé WA, Fenwick JD, et al. A challenge to traditional radiation oncology. Int J Radiat Oncol Biol Phys 2004;60:1241-56. [PubMed]
- Hof H, Herfarth KK, Münter M, et al. Stereotactic single-dose radiotherapy of stage I non-small-cell lung cancer (NSCLC). Int J Radiat Oncol Biol Phys 2003;56:335-41. [PubMed]
- Uematsu M, Shioda A, Tahara K, et al. Focal, high dose, and fractionated modified stereotactic radiation therapy for lung carcinoma patients: a preliminary experience. Cancer 1998;82:1062-70. [PubMed]
- Li B, Yu J, Suntharalingam M, et al. Comparison of three treatment options for single brain metastasis from lung cancer. Int J Cancer 2000;90:37-45. [PubMed]
- Rades D, Pluemer A, Veninga T, et al. Whole-brain radiotherapy versus stereotactic radiosurgery for patients in recursive partitioning analysis classes 1 and 2 with 1 to 3 brain metastases. Cancer 2007;110:2285-92. [PubMed]
- Wang LG, Guo Y, Zhang X, et al. Brain metastasis: experience of the Xi-Jing hospital. Stereotact Funct Neurosurg 2002;78:70-83. [PubMed]
- Papiez L, Timmerman R. Hypofractionation in radiation therapy and its impact. Med Phys 2008;35:112-8. [PubMed]
- de Pooter JA, Méndez Romero A, Jansen WP, et al. Computer optimization of noncoplanar beam setups improves stereotactic treatment of liver tumors. Int J Radiat Oncol Biol Phys 2006;66:913-22. [PubMed]
- de Pooter JA, Méndez Romero A, Wunderink W, et al. Automated non-coplanar beam direction optimization improves IMRT in SBRT of liver metastasis. Radiother Oncol 2008;88:376-81. [PubMed]
- Liu R, Buatti JM, Howes TL, et al. Optimal number of beams for stereotactic body radiotherapy of lung and liver lesions. Int J Radiat Oncol Biol Phys 2006;66:906-12. [PubMed]
- Chang BK, Timmerman RD. Stereotactic body radiation therapy: a comprehensive review. Am J Clin Oncol 2007;30:637-44. [PubMed]
- Blomgren H, Lax I, Näslund I, et al. Stereotactic high dose fraction radiation therapy of extracranial tumors using an accelerator. Clinical experience of the first thirty-one patients. Acta Oncol 1995;34:861-70. [PubMed]
- Lohr F, Debus J, Frank C, et al. Noninvasive patient fixation for extracranial stereotactic radiotherapy. Int J Radiat Oncol Biol Phys 1999;45:521-7. [PubMed]
- Herfarth KK, Debus J, Lohr F, et al. Stereotactic single-dose radiation therapy of liver tumors: results of a phase I/II trial. J Clin Oncol 2001;19:164-70. [PubMed]
- Uematsu M, Shioda A, Suda A, et al. Computed tomography-guided frameless stereotactic radiotherapy for stage I non-small cell lung cancer: a 5-year experience. Int J Radiat Oncol Biol Phys 2001;51:666-70. [PubMed]
- Haasbeek CJ, Slotman BJ, Senan S. Radiotherapy for lung cancer: clinical impact of recent technical advances. Lung Cancer 2009;64:1-8. [PubMed]
- Gui M, Feng Y, Yi B, et al. Four-dimensional intensity-modulated radiation therapy planning for dynamic tracking using a direct aperture deformation (DAD) method. Med Phys 2010;37:1966-75. [PubMed]
- Liu HH, Balter P, Tutt T, et al. Assessing Respiration-Induced Tumor Motion and Internal Target Volume Using Four-Dimensional Computed Tomography for Radiotherapy of Lung Cancer. Int J Radiat Oncol Biol Phys 2007;68:531-40. [PubMed]
- Seppenwoolde Y, Shirato H, Kitamura K, et al. Precise and real-time measurement of 3D tumor motion in lung due to breathing and heartbeat, measured during radiotherapy. Int J Radiat Oncol Biol Phys 2002;53:822-34. [PubMed]
- Keall P. 4-dimensional computed tomography imaging and treatment planning. Semin Radiat Oncol 2004;14:81-90. [PubMed]
- Briere TM, Beddar S, Balter P, et al. Respiratory gating with EPID-based verification: the MDACC experience. Phys Med Biol 2009;54:3379-91. [PubMed]
- Hoisak JD, Sixel KE, Tirona R, et al. Correlation of lung tumor motion with external surrogate indicators of respiration. Int J Radiat Oncol Biol Phys 2004;60:1298-306. [PubMed]
- Keall PJ, Cattell H, Pokhrel D, et al. Geometric accuracy of a real-time target tracking system with dynamic multileaf collimator tracking system. Int J Radiat Oncol Biol Phys 2006;65:1579-84. [PubMed]
- Guckenberger M, Meyer J, Wilbert J, et al. Precision of image-guided radiotherapy (IGRT) in six degrees of freedom and limitations in clinical practice. Strahlenther Onkol 2007;183:307-13. [PubMed]
- Boda-Heggemann J, Fleckenstein J, Lohr F, et al. Multiple breath-hold CBCT for online image guided radiotherapy of lung tumors: simulation with a dynamic phantom and first patient data. Radiother Oncol 2011;98:309-16. [PubMed]
- Dawson LA, Eccles C, Bissonnette JP, et al. Accuracy of daily image guidance for hypofractionated liver radiotherapy with active breathing control. Int J Radiat Oncol Biol Phys 2005;62:1247-52. [PubMed]
- Panakis N, McNair HA, Christian JA, et al. Defining the margins in the radical radiotherapy of non-small cell lung cancer (NSCLC) with active breathing control (ABC) and the effect on physical lung parameters. Radiother Oncol 2008;87:65-73. [PubMed]
- Fakiris AJ, McGarry RC, Yiannoutsos CT, et al. Stereotactic body radiation therapy for early-stage non-small-cell lung carcinoma: four-year results of a prospective phase II study. Int J Radiat Oncol Biol Phys 2009;75:677-82. [PubMed]
- Baumann P, Nyman J, Hoyer M, et al. Outcome in a prospective phase II trial of medically inoperable stage I non-small-cell lung cancer patients treated with stereotactic body radiotherapy. J Clin Oncol 2009;27:3290-6. [PubMed]
- Timmerman R, McGarry R, Yiannoutsos C, et al. Excessive toxicity when treating central tumors in a phase II study of stereotactic body radiation therapy for medically inoperable early-stage lung cancer. J Clin Oncol 2006;24:4833-9. [PubMed]
- Koto M, Takai Y, Ogawa Y, et al. A phase II study on stereotactic body radiotherapy for stage I non-small cell lung cancer. Radiother Oncol 2007;85:429-34. [PubMed]
- Ricardi U, Filippi AR, Guarneri A, et al. Stereotactic body radiation therapy for early stage non-small cell lung cancer: results of a prospective trial. Lung Cancer 2010;68:72-7. [PubMed]
- Timmerman R, Paulus R, Galvin J, et al. Stereotactic body radiation therapy for inoperable early stage lung cancer. JAMA 2010;303:1070-6. [PubMed]
- Boda-Heggemann J, Lohr F, Wertz H, et al. Repeat ABC breath- hold Imaging With Cone-beam CT. ASTRO 2011 Abstract 2010.
- De Neve W, De Wagter C. Lethal pneumonitis in a phase I study of chemotherapy and IMRT for NSCLC: the need to investigate the accuracy of dose computation. Radiother Oncol 2005;75:246-7. [PubMed]
- Voroney JP, Hope A, Dahele MR, et al. Chest wall pain and rib fracture after stereotactic radiotherapy for peripheral non-small cell lung cancer. J Thorac Oncol 2009;4:1035-7. [PubMed]
- Song SY, Choi W, Shin SS, et al. Fractionated stereotactic body radiation therapy for medically inoperable stage I lung cancer adjacent to central large bronchus. Lung Cancer 2009;66:89-93. [PubMed]
- Guckenberger M, Heilman K, Wulf J, et al. Pulmonary injury and tumor response after stereotactic body radiotherapy (SBRT): results of a serial follow-up CT study. Radiother Oncol 2007;85:435-42. [PubMed]
- Kirkpatrick JP, Meyer JJ, Marks LB. The linear-quadratic model is inappropriate to model high dose per fraction effects in radiosurgery. Semin Radiat Oncol 2008;18:240-3. [PubMed]
- Garcia-Barros M, Paris F, Cordon-Cardo C, et al. Tumor response to radiotherapy regulated by endothelial cell apoptosis. Science 2003;300:1155-9. [PubMed]
- Fuks Z, Kolesnick R. Engaging the vascular component of the tumor response. Cancer Cell 2005;8:89-91. [PubMed]
- Paris F, Fuks Z, Kang A, et al. Endothelial apoptosis as the primary lesion initiating intestinal radiation damage in mice. Science 2001;293:293-7. [PubMed]
- Truman JP, García-Barros M, Kaag M, et al. Endothelial membrane remodeling is obligate for anti-angiogenic radiosensitization during tumor radiosurgery. PLoS One 2010;5:e12310. [PubMed]
- Bao S, Wu Q, McLendon RE, et al. Glioma stem cells promote radioresistance by preferential activation of the DNA damage response. Nature 2006;444:756-60. [PubMed]
- Al-Hajj M, Wicha MS, Benito-Hernandez A, Morrison SJ, Clarke MF. Prospective identification of tumorigenic breast cancer cells. Proc Natl Acad Sci USA 2003;100:3983-8. [PubMed]
- Ch'ang HJ, Maj JG, Paris F, et al. ATM regulates target switching to escalating doses of radiation in the intestines. Nat Med 2005;11:484-90. [PubMed]
- McBride WH, Chiang CS, Olson JL, et al. A sense of danger from radiation. Radiat Res 2004;162:1-19. [PubMed]
- Demaria S, Formenti SC. Sensors of ionizing radiation effects on the immunological microenvironment of cancer. Int J Radiat Biol 2007;83:819-25. [PubMed]
- Apetoh L, Ghiringhelli F, Tesniere A, Obeid M, Ortiz C, Criollo A, et al. Toll-like receptor 4-dependent contribution of the immune system to anticancer chemotherapy and radiotherapy. Nat Med 2007;13:1050-9. [PubMed]
- Yeung AR, Li JG, Shi W, et al. Tumor localization using cone-beam CT reduces setup margins in conventionally fractionated radiotherapy for lung tumors. Int J Radiat Oncol Biol Phys 2009;74:1100-7. [PubMed]
- Boda-Heggemann J, Lohr F, Wenz F, Flentje M, et al. kV cone-beam CT-based IGRT: a clinical review. Strahlenther Onkol 2011;187:284-91. [PubMed]
- Wang L, Feigenberg S, Chen L, et al. Benefit of three-dimensional image-guided stereotactic localization in the hypofractionated treatment of lung cancer. Int J Radiat Oncol Biol Phys 2006;66:738-47. [PubMed]
- Oita M, Takegawa Y, Yagi H, et al. Nihon Hoshasen Gijutsu Gakkai Zasshi 2006;62:711-3. [PubMed]
- Amer A, Marchant T, Sykes J, et al. Imaging doses from the Elekta Synergy X-ray cone beam CT system. Br J Radiol 2007;80:476-82. [PubMed]
- Roxby P, Kron T, Foroudi F, et al. Simple methods to reduce patient dose in a Varian cone beam CT system for delivery verification in pelvic radiotherapy. Br J Radiol 2009;82:855-9. [PubMed]
- Groh BA, Siewerdsen JH, Drake DG, et al. A performance comparison of flat-panel imager-based MV and kV cone-beam CT. Med Phys 2002;29:967-75. [PubMed]
- Fuss M, Salter BJ, Cavanaugh SX, et al. Daily ultrasound-based image-guided targeting for radiotherapy of upper abdominal malignancies. Int J Radiat Oncol Biol Phys 2004;59:1245-56. [PubMed]
- Boda-Heggemann J, Mennemeyer P, Wertz H, et al. Accuracy of ultrasound-based image guidance for daily positioning of the upper abdomen: an online comparison with cone beam CT. Int J Radiat Oncol Biol Phys 2009;74:892-7. [PubMed]
- Kupelian P, Willoughby T, Mahadevan A, et al. Multi-institutional clinical experience with the Calypso System in localization and continuous, real-time monitoring of the prostate gland during external radiotherapy. Int J Radiat Oncol Biol Phys 2007;67:1088-98. [PubMed]
- Wertz H, Lohr F, Dobler B, et al. Dosimetric consequences of a translational isocenter correction based on image guidance for intensity modulated radiotherapy (IMRT) of the prostate. Phys Med Biol 2007;52:5655-65. [PubMed]
- Wertz H, Lohr F, Dobler B, et al. Strahlenther Onkol 2007;183:203-10. [PubMed]
- Walter C, Boda-Heggemann J, Wertz H, et al. In vivo dose measurements of extra dose from cone-beam computed tomography. Int J Radiat Oncol Biol Phys 2006;66:632. [PubMed]
- Juhler Nøttrup T, Korreman SS, Pedersen AN, et al. Intra- and interfraction breathing variations during curative radiotherapy for lung cancer. Radiother Oncol 2007;84:40-8. [PubMed]
- Rietzel E, Pan T, Chen GT. Four-dimensional computed tomography: image formation and clinical protocol. Med Phys 2005;32:874-89. [PubMed]
- Sonke JJ, Rossi M, Wolthaus J, et al. Frameless stereotactic body radiotherapy for lung cancer using four-dimensional cone beam CT guidance. Int J Radiat Oncol Biol Phys 2009;74:567-74. [PubMed]
- Underberg RW, Lagerwaard FJ, Slotman BJ, et al. Benefit of respiration-gated stereotactic radiotherapy for stage I lung cancer: an analysis of 4DCT datasets. Int J Radiat Oncol Biol Phys 2005;62:554-60. [PubMed]
- Barnes EA, Murray BR, Robinson DM, et al. Dosimetric evaluation of lung tumor immobilization using breath hold at deep inspiration. Int J Radiat Oncol Biol Phys 2001;50:1091-8. [PubMed]
- Kashani R, Balter JM, Hayman JA, et al. Short-term and long-term reproducibility of lung tumor position using active breathing control (ABC). Int J Radiat Oncol Biol Phys 2006;65:1553-9. [PubMed]
- Partridge M, Tree A, Brock J, et al. Improvement in tumour control probability with active breathing control and dose escalation: a modelling study. Radiother Oncol 2009;91:325-9. [PubMed]
- Thompson BP, Hugo GD. Quality and accuracy of cone beam computed tomography gated by active breathing control. Med Phys 2008;35:5595-608. [PubMed]
- Blessing M, Stsepankou D, Wertz H, et al. Breath-hold target localization with simultaneous kilovoltage/megavoltage cone-beam computed tomography and fast reconstruction. Int J Radiat Oncol Biol Phys 2010;78:1219-26. [PubMed]
- Wertz H, Stsepankou D, Blessing M, et al. Fast kilovoltage/megavoltage (kVMV) breathhold cone-beam CT for image-guided radiotherapy of lung cancer. Phys Med Biol 2010;55:4203-17. [PubMed]